Continuous Seismic Profiling
Basic Concept
Continuous seismic profiling (CSP) is a waterborne seismic reflection technique that can be employed on a variety of waterbodies. CSP methods can map the depth to water bottom and, under ideal conditions, delineate the structures of underlying sub-bottom sediments and/or bedrock. Imaging sub-bottom layers (i.e., multi-layered earth materials beneath the water bottom) using CSP requires certain environmental conditions and physical parameters. Results also are dependent on the instrumentation used and the geologic complexity.
Successful CSP data collection involves the transmission of seismic energy through the water column into the subsurface to, with a high-enough frequency, resolve any sub-bottom layers. CSP has the potential to differentiate materials that have a sharp and sufficient acoustic impedance contrast. Given such, CSP data have proven useful in numerous environmental studies that involve the characterization and differentiation of lithology and identification of hydrogeologic boundaries (Johnson and White, 2007).
Theory
The CSP method transmits acoustic-energy signals (i.e., elastic waves) that travel into and through the water column. A portion of the signal energy is absorbed by the water column, while another is reflected back by the water bottom. The remaining energy penetrates the subsurface, propagates through the sub-bottom materials, and can reflect off material interfaces due to acoustic-impedance contrasts. The reflected energy is detected by receivers that are towed along the water surface (Kress and others, 2004).
Thus, the water bottom and subsurface-sediment interfaces can behave as acoustic boundaries that reflect signal energy back to the CSP receiver(s). Acoustic boundaries occur at interfaces of contrasting acoustic impedance, which is a material property defined as the product of seismic velocity and density (i.e., Z = ρ V). The densities of shallow sub-bottom materials are generally similar, and, thus, reflections occur mostly because of changes in the seismic velocity of the materials (Trabant and others, 1997).
The strength of the reflected signal is described by the reflection coefficient (R), which is related to the acoustic impedance contrast magnitude. Material interfaces with higher acoustic-impedance contrasts have larger reflection coefficients, whereas those with lower contrasts are more likely to allow signal propagation without reflections. Interfaces with R ≅ 1 (e.g., water-to-air, water-to-bedrock) are described as strong reflectors, and those with R ≅ 0 (e.g., water-to-mud, mud-to-sediments) are weakly reflective (Johnson and White, 2007; Sylwester, 1983).
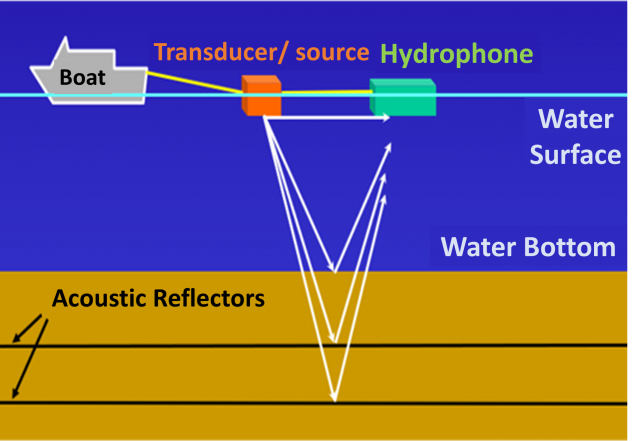
(source USGS)
Among other factors (e.g., source power, signal frequency, particle interactions, etc.), signal penetration into the sub-bottom depends heavily upon the water-bottom reflection coefficient. Because they are strong reflectors, seismically “hard” water bottoms often inhibit signal propagation and reflect most of the signal back to the water surface. Multiple reflections within seismic sections result from the returning signal reflecting off the water-air interface and reverberating continuously between these two hard interfaces. (Cardinell and others, 1990; Johnson and White, 2007)
Seismically hard water bottoms prevent signal penetration and obscure the imaging of sub-bottom reflectors. Hard water bottoms include rock, cobbles, engineered/compacted surfaces, and/or entrapped gas. There are no adjustments that can be made to the data collection to overcome a seismically hard water bottom. Although, if the reflection is off gas, it may be advantageous to collect the data during colder temperatures when organic activity is lower.
Subsurface-reflector depths can be calculated using the two-way travel times (TWT) of the seismic waves and seismic-velocity values of the earth materials. The time between when the signal is transmitted and received (i.e., TWT data) is measured. Seismic velocities (i.e., media-specific speed of sound) are assumed or computed from a control point with known depth. CSP typically estimates the seismic velocity of the water and sub-bottom sediments at 5,000 feet per second for freshwater (Placzek and Haeni, 1995).
Applications
A CSP survey is conducted using a watercraft that is piloted along predetermined and/or georeferenced transects (see the picture below). The sound source (e.g., transmitter, transducer, boomer-plate, or sparker) is either hull-mounted or towed. The returning signals are measured with a single receiver or streamer (i.e., receiver string) in tow. CSP data collection is monitored and controlled using an onboard computer and control unit, and data are displayed live, which allows for real-time survey adjustments.
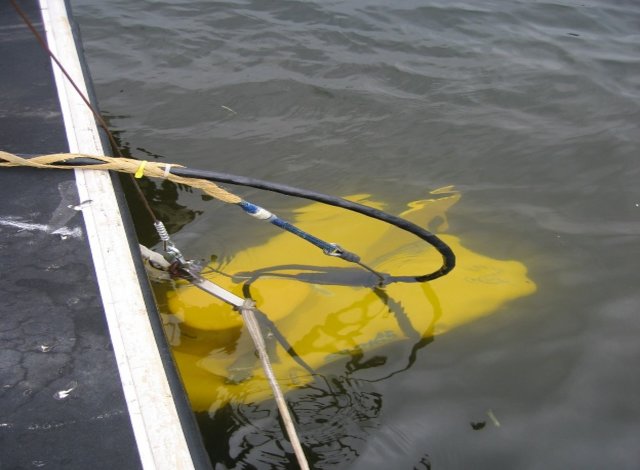
(source USGS)
As a seismic wave travels through the subsurface, signal energy is attenuated (i.e., lost) through numerous interactions at a rate that is related to wave frequency. High-frequency waves attenuate more readily than lower-frequency waves. Consequently, low-frequency signals have an increased depth of penetration, though they render images with lower resolution. Conversely, higher-frequency signals have increased ability to resolve smaller features, and, therefore, there is a tradeoff when using a single-frequency signal (Johnson and White, 2007).
Thus, the sound source commonly employed in CSP is a CHIRP (i.e., Compressed High Intensity Radar Pulse) or swept frequency. In such systems, the CSP transmitter digitally generates a linear, frequency-modulated acoustic signal pulse that sweeps over a user-specified frequency range. The benefits of using a CHIRP source is the combination of the greater penetration potential of lower frequency signals and the increased resolution of the higher frequencies (Kress and others, 2004).
Using reflection coefficient, seismic velocity, attenuation, and reflection-signature information, sediment classification can be estimated. For example, a buried gravel might have increased attenuation, increased velocity, and point reflectors (i.e., pebbles) (LeBlanc and others, 1992). However, CSP data alone cannot explicitly determine hydrogeologic-unit identities, quantitative hydraulic parameters, or depth. Further data or constraints are needed to confirm any interpretations of CSP data, which under ideal equipment and earth conditions, have successfully aided the following:
- Determination of water-bottom surface
- Delineation of the depth to sub-bottom layers/interfaces (e.g., bedrock)
- Characterization of sub-bottom layers/interfaces
- Characterization of stratigraphy and classification of sediment
- Identification of sedimentation and inundation/infilling
- Removal of dams and erosion concerns
- Detection of cavities
- Identification of trapped gasses and organic material
Examples/Case studies
Cardinell, A.P., Harned, D.A., and Berg, S.A., 1990, Continuous seismic reflection profiling of hydrogeologic features beneath New River, Camp Lejeune, North Carolina: U.S. Geological Survey Water-Resources Investigations Report 89-4195, 33 p., doi:10.3133/wri894195.
Abstract: Continuous seismic reflection profiling of the New River within the Camp Lejeune Marine Corps Base was conducted to collect data for use in describing the structural and depositional setting of the surficial and Castle Hayne aquifers and other hydrogeologic units underlying the New River. The Castle Hayne aquifer serves as the principal water supply for
the Base. A medium-power, wide-frequency seismic system was used to collect more than 100 miles of continuous s ingle-channel, shallow-water seismic reflection records in the study area over a 4-day period in December 1987. Positioning of the seismic boat was controlled by an onboard integrated navigation system throughout the survey. LORAN C and Global Positioning
Systems were integrated to plot position during the profiling. The Castle Hayne aquifer and deeper aquifers show continuous reflectors throughout the study reach and gently dip to the southeast. There is no evidence of faulting across the New River. The younger, shallower reflectors generally are undifferentiated and lack the lateral continuity of the underlying older reflectors. Paleochannels of Quaternary and Tertiary age were found at several locations and depths in the New River. If paleochannels filled with permeable material exist beneath the present land surface, these features could act as conduits for ground-water flow or movement of contaminants between the surficial and Castle Hayne aquifers.
Haeni, F.P., 1996, Use of Ground‐Penetrating Radar and Continuous Seismic‐Reflection Profiling on Surface‐Water Bodies in Environmental and Engineering Studies: Journal of Environmental and Engineering Geophysics, v. 1., no. 1, p. 1-84, doi:10.4133/JEEG1.1.27.
Abstract: Ground‐penetrating radar (GPR) and continuous seismic‐reflection profiling (CSP) on shallow rivers, lakes, and ponds are efficient and economical ways of obtaining subsurface hydrologic and geologic information for environmental and engineering studies. These methods are similar in that they produce continuous subsurface profiles, are easy to use in some applications, and the records can occasionally be straightforward to interpret. They are dissimilar in that GPR cannot penetrate electrically conductive water or subsurface sediments, and CSP usually cannot operate in water less than 5 feet (ft) deep. GPR records collected on a lake in New Hampshire have been interpreted to estimate the depth to bedrock and to evaluate the grain‐size characteristics of the underlying stratified drift at the lakeshore boundary. In a pond in Massachusetts, CSP and GPR were used to determine depth to bedrock and the grain‐size characteristics of the subbottom materials in part of the pond. Water‐column multiple reflections, depth and conductivity of water and subsurface materials, and diffractions degraded the quality of the GPR records. CSP records collected in the Connecticut River near Hartford, Connecticut were used to estimate the depth of till and bedrock interfaces and to evaluate grain‐size characteristics of subsurface materials. Interpreted CSP records also can indicate bedding planes within consolidated rock units. Water‐column multiple reflections and very shallow water degraded the quality of the CSP records. GPR and CSP methods have been used to delineate infilled scour holes near bridge piers. Scour holes that were filled with up to 8 ft. of loose sand were mapped during engineering scour studies near a bridge in Connecticut. Because GPR and CSP operate on different physical principles, the two geophysical methods complement each other. Depending on the required depth of penetration and the degree of resolution needed, one or both of these methods can be used to acquire accurate and reliable subsurface hydrologic and geologic information critical to environmental and engineering studies.
Johnson, C.D., and White, E.A., 2007, Marine geophysical investigation of selected sites in Bridgeport Harbor, Connecticut, 2006: U.S. Geological Survey Scientific Investigations report 2007-5119, 32 p., doi:10.3133/SIR20075119.
Abstract: A marine geophysical investigation was conducted in 2006 to help characterize the bottom and subbottom materials and extent of bedrock in selected areas of Bridgeport Harbor, Connecticut. The data will be used by the U.S. Army Corps of Engineers in the design of confined aquatic disposal (CAD) cells within the harbor to facilitate dredging of the harbor. Three water-based geophysical methods were used to evaluate the geometry and composition of subsurface materials: (1) continuous seismic profiling (CSP) methods provide the depth to water bottom, and when sufficient signal penetration can be achieved, delineate the depth to bedrock and subbottom materials; (2) continuous resistivity profiling (CRP) methods were used to define the electrical properties of the shallow subbottom, and to possibly determine the distribution of conductive materials, such as clay, and resistive materials, such as sand and bedrock; (3) and magnetometer data were used to identify conductive anomalies of anthropogenic sources, such as cables and metallic debris. All data points were located using global positioning systems (GPS), and the GPS data were used for real-time navigation. The results of the CRP, CSP, and magnetometer data are consistent with the conceptual site model of a bedrock channel incised beneath the present day harbor. The channel appears to follow a north-northwest to south-southeast trend and is parallel to the Pequannock River. The seismic record and boring data indicate that under the channel, the depth to bedrock is as much as 42.7 meters (m) below mean low-low water (MLLW) in the dredged part of the harbor. The bedrock channel becomes shallower towards the shore, where bedrock outcrops have been mapped at land surface. CSP and CRP data were able to provide a discontinuous, but reasonable, trace from the channel toward the west under the proposed southwestern CAD cell. The data indicate a high amount of relief on the bedrock surface, as well as along the water bottom. Under the southwestern CAD cell, the sediments are only marginally thick enough for a CAD cell, at about 8 to 15 m in depth. Some of the profiles show small diffractions in the unconsolidated sediments, but no large-scale boulders or boulder fields were identified. No bedrock reflectors were imaged under the southeastern CAD cell, where core logs indicate the rock is as much as 30 m below MLLW. The chirp frequency, tuned transducer, and boomer-plate CSP surveys were adversely affected by a highly reflective water bottom causing strong multiples in the seismic record and very limited depths of penetration. These multiples are attributed to entrapped gas (methane) in the sediments or to very hard bottom conditions. In a limited number of places, the bedrock surface was observed in the CSP record, creating a discontinuous and sporadic image of the bedrock surface. These interpretations generally matched core data at FP-03-10 and FB-06-1. Use of two analog CSP systems, the boomer plate and tuned transducer, did not overcome the reflections off the water bottom and did not improve the depth of penetration. In general, the CRP profiles were used to corroborate the results of the CSP profiles. Relatively resistive zones associated with the locations of seismic reflections were interpreted as bedrock. The shape of the bedrock surface generally was similar in the CRP and CSP profiles. Evaluation of the CRP profiles indicated that the inversions were adversely affected where the depth and (or) ionic concentration of the water column varied. Consequently, the CRP profiles were broken into short intervals that extended just over the area of interest, where the depth to water bottom was fairly constant. Over these short profiles, efforts were made to evaluate the resistivity of the very shallow sediments to determine if there were any large contrasts in the resistivity of the sediments that might indicate differences in the shallow subbottom materials. No conclusions about the overburden lithology, however, can be drawn from the distribution of resistivity in the profiles. A series of magnetic surveys also were conducted in Bridgeport Harbor. The magnetic data from Profiles 3, A, and B show a significant magnetic anomaly trending northeast and southwest. These anomalies indicate a possible large-scale feature that may be anthropogenic. Other isolated anomalies did not appear to be continuous or large scale. These features could be related to topographic changes (and changes in the height of the sensor above the bottom) or to metallic debris on the bottom or in the subbottom.
Jones, P.M., Trost, J.J., Diekoff, A.L., Rosenberry, D.O., White, E.A., Erickson, M.L., Morel, D.L., and Heck, J.M., 2016, Statistical analysis of lake levels and field study of groundwater and surface-water exchanges in the northeast Twin Cities Metropolitan Area, Minnesota, 2002 through 2015: U.S. Geological Survey Scientific Investigations report 2016-5139-A, 86 p., doi:10.3133/sir20165139A.
Abstract: Water levels declined from 2003 to 2011 in many lakes in Ramsey and Washington Counties in the northeast Twin Cities Metropolitan Area, Minnesota; however, water levels in other northeast Twin Cities Metropolitan Area lakes increased during the same period. Groundwater and surface-water exchanges can be important in determining lake levels where these exchanges are an important component of the water budget of a lake. An understanding of groundwater and surface-water exchanges in the northeast Twin Cities Metropolitan Area has been limited by the lack of hydrologic data. The U.S. Geological Survey, in cooperation with the Metropolitan Council and Minnesota Department of Health, completed a field and statistical study assessing lake-water levels and regional and local groundwater and surface-water exchanges near northeast Twin Cities Metropolitan Area lakes. This report documents the analysis of collected hydrologic, water-quality, and geophysical data; and existing hydrologic and geologic data to (1) assess the effect of physical setting and climate on lake-level fluctuations of selected lakes, (2) estimate potential percentages of surface-water contributions to well water across the northeast Twin Cities Metropolitan Area, (3) estimate general ages for waters extracted from the wells, and (4) assess groundwater inflow to lakes and lake-water outflow to aquifers downgradient from White Bear Lake.
Statistical analyses of lake levels during short-term (2002–10) and long-term (1925–2014) periods were completed to help understand lake-level changes across the northeast Twin Cities Metropolitan Area. Comparison of 2002–10 lake levels to several landscape and geologic characteristics explained variability in lake-level changes for 96 northeast Twin Cities Metropolitan Area lakes. Application of several statistical methods determined that (1) closed-basin lakes (without an active outlet) had larger lake-level declines than flow-through lakes with an outlet; (2) closed-basin lake-level changes reflected groundwater-level changes in the Quaternary, Prairie du Chien, and Jordan aquifers; (3) the installation of outlet-control structures, such as culverts and weirs, resulted in smaller multiyear lake-level changes than lakes without outlet-control structures; (4) water levels in lakes primarily overlying Superior Lobe deposits were significantly more variable than lakes primarily overlying Des Moines Lobe deposits; (5) lake-level declines were larger with increasing mean lake-level elevation; and (6) the frequency of some of these characteristics varies by landscape position. Flow-through lakes and lakes with outlet-control structures were more common in watersheds with more than 50 percent urban development compared to watersheds with less than 50 percent urban development. A comparison of two 35-year periods during 1925–2014 revealed that variability of annual mean lake levels in flow-through lakes increased when annual precipitation totals were more variable, whereas variability of annual mean lake levels in closed-basin lakes had the opposite pattern, being more variable when annual precipitation totals were less variable.
Oxygen-18/oxygen-16 and hydrogen-2/hydrogen-1 ratios for water samples from 40 wells indicated the well water was a mixture of surface water and groundwater in 31 wells, whereas ratios from water sampled from 9 other wells indicated that water from these wells receive no surface-water contribution. Of the 31 wells with a mixture of surface water and groundwater, 11 were downgradient from White Bear Lake, likely receiving water from deeper parts of the lake.
Age dating of water samples from wells indicated that the age of water in the Prairie du Chien and Jordan aquifers can vary widely across the northeast Twin Cities Metropolitan Area. Estimated ages of recharge for 9 of the 40 wells sampled for chlorofluorocarbon concentrations ranged widely from the early 1940s to mid-1970s. The wide range in estimated ages of recharge may have resulted from the wide range in the open-interval lengths and depths for the wells.
Results from stable isotope analyses of water samples, lake-sediment coring, continuous seismic-reflection profiling, and water-level and flow monitoring indicated that there is groundwater inflow from nearshore sites and lake-water outflow from deep-water sites in White Bear Lake. Continuous seismic-reflection profiling indicated that deep sections of White Bear, Pleasant, Turtle, and Big Marine Lakes have few trapped gases and little organic material, which indicates where groundwater and lake-water exchanges are more likely. Water-level differences between White Bear Lake and piezometer and seepage measurements in deep waters of the lake indicate that groundwater and lake-water exchange is happening in deep waters, predominantly downgradient from the lake and into the lake sediment. Seepage fluxes measured in the nearshore sites of White Bear Lake generally were higher than seepage fluxes measured in the deep-water sites, which indicates that groundwater-inflow rates at most of the nearshore sites are higher than lake-water outflow from the deep-water sites.
Kress, W.H., Dietsch, B.J., Steele, G.V., White, E.A., and Cannia, J.C., 2004, Use of Continuous Seismic Profiling to Differentiate Geologic Deposits Underlying Selected Canals in Central and Western Nebraska: U.S. Geological Survey Fact Sheet 115-03, 6 p., doi:10.3133/fs11503.
Abstract: In central and western Nebraska, the Platte River and its underlying alluvial aquifer are vital sources of water for irrigation, power production, and recreation. Scientists and water managers need accurate knowledge of the saturated thickness, or thickness between the water table and less permeable underlying bedrock, of the alluvial aquifer to better understand ground-water movement and interactions between ground water and surface water (COHYST Technical Committee, 2000). Typically geologic logs and bedrock outcrops are used to map the depth of the bedrock surface. Although geophysical methods have been used to investigate shallow geologic deposits in central and western Nebraska (Ellis and Hiergesell, 1985), no investigations had been conducted using water-borne continuous seismic-reflection profiling (CSP) techniques. In 2001, the U.S. Geological Survey (USGS) and the Nebraska Platte River Cooperative Hydrology Study (COHYST) engaged in a cooperative pilot study to determine if CSP could be used to determine depth to bedrock and the general characteristics of alluvial sediments in the Platte River Basin (fig. 1). This report: (1) describes the methodology used to obtain the CSP records, (2) provides examples of data obtained from CSP surveys, and (3) evaluates the usefulness of the results obtained with the CSP technique.
Taylor, D.I., 1992, Nearshore shallow gas around the U.K. coast: Continental Shelf Research, v. 12, no. 10, p. 1135-1144, doi:10.1016/0278-4343(92)90074-T.
Abstract: The occurrence of shallow gas in seafloor sediments within 50 km of the coast of the British Isles is generally attributed to the degradation of organic matter within post glacial deposits. However there is evidence that buried rockhead topography may also be a factor governing the existence of shallow gas in some localities. In such cases gas may be escaping from the rock through fissures. Where channels have been cut into the rockhead and are now filled with unconsolidated sediments, gas often accumulates in the central portion of the channels. This may be due to the greater thickness of sediment in the centre of the channels generating sufficient gas to be detectable in seismic profiling data. The process of compaction causing gas to be squeezed out of the pore spaces may also be a contributory factor. In other localities no such relationship appears to exist and the gas is present at very shallow levels (within 5 m of the seabed) in the superficial sediment column. Detailed analyses of high resolution continuous seismic reflection profiles from Cardigan Bay, the northeast Irish Sea and the Firth of Forth, would appear to permit the categorization of nearshore shallow gas zones around these parts of the U.K. continental shelf and probably elsewhere, in order to assist with the explanation of their occurrence and the likely hazard that the zones present to seabed and sub-seabed structures.
Vrbancich, J., Whiteley, R.J., Caffi, P., and Emerson, D.W., 2011, Marine seismic profiling and shallow marine sand resistivity investigations in Broken Bay, NSW, Australia: Exploration Geophysics, v. 42, no. 4, p. 227-238, doi:10.1071/EG11031.
Abstract: A marine continuous seismic profiling (CSP) study and a resistivity study of vibrocore samples of shallow marine sands were undertaken in Broken Bay, NSW, Australia, to characterise the seabed. The overall aims were to provide an estimate of the sediment thickness using CSP and an estimate of sediment resistivity in the upper 4 m of the seabed at selected sites. This information can provide a simplified geo-electrical model to assess the accuracy of interpreted seabed and bedrock depths obtained independently from AEM data and to assist the calibration of AEM instrumentation for bathymetric surveys. The acoustic impedance contrast between deeper sediments and the anticipated basement sandstone was variable suggesting irregularly layered sediments. These sediments contained very dense (tightly packed) sands, owing to marine regression and transgression of sea level, and variably weathered underlying sandstones. Interpreted bedrock levels vary considerably across the survey area and represent drowned river valleys of the inner continental shelf. A broad deep channel representing a high- energy paleo-fluvial drainage system in the Hawkesbury River outreaches was identified; it extended to approximately –80 m AHD. Another area revealed a dendritic fluvial pattern extending to approximately –70 m AHD. A moderately narrow palaeovalley extending to–90 m AHD either side of the Palm Beach tombolo was clearly identified. This same feature was also clearly identified from airborne electromagnetic data which, when interpreted, showed very good agreement with seismic depths. Sand samples from 17 sites were obtained from vibrocores with a penetration depth of 4.5 m. The laboratory resistivity values, obtained from four-electrode measurements on 64 seawater saturated subsamples ranged from 1.3 to 0.6 Ωm (20 °C); 0.92 Ωm average. Inter- and intra-site changes in composition (shell/sand), grain packing, grain size, grain shape, cohesion, and inferred porosity were thought to be responsible for minor variations in resistivity. Archie Equation plots showed some scatter, but the data indicate a cementation factor of ~1.6 and an average formation factor of 4.2 for the suites of sands. These values are consistent with values cited in the literature for similar lithologies.
References
Cardinell, A.P., Harned, D.A., and Berg, S.A., 1990, Continuous seismic reflection profiling of hydrogeologic features beneath New River, Camp Lejeune, North Carolina: U.S. Geological Survey Water-Resources Investigations Report 89-4195, 33 p., doi:10.3133/wri894195.
Haeni, F.P., 1986, Application of continuous seismic-reflection methods to hydrologic studies: Ground Water, v. 24, no. 1, p. 23-31, doi:10.1111/j.1745-6584.1986.tb01455.x.
Haeni, F.P., 1996, Use of Ground‐Penetrating Radar and Continuous Seismic‐Reflection Profiling on Surface‐Water Bodies in Environmental and Engineering Studies: Journal of Environmental and Engineering Geophysics, v. 1., no. 1, p. 1-84, doi:10.4133/JEEG1.1.27.
Johnson, C.D., and White, E.A., 2007, Marine geophysical investigation of selected sites in Bridgeport Harbor, Connecticut, 2006: U.S. Geological Survey Scientific Investigations report 2007-5119, 32 p., doi:10.3133/SIR20075119.
Jones, P.M., Trost, J.J., Diekoff, A.L., Rosenberry, D.O., White, E.A., Erickson, M.L., Morel, D.L., and Heck, J.M., 2016, Statistical analysis of lake levels and field study of groundwater and surface-water exchanges in the northeast Twin Cities Metropolitan Area, Minnesota, 2002 through 2015: U.S. Geological Survey Scientific Investigations report 2016-5139-A, 86 p., doi:10.3133/sir20165139A.
Kress, W.H., Dietsch, B.J., Steele, G.V., White, E.A., and Cannia, J.C., 2004, Use of Continuous Seismic Profiling to Differentiate Geologic Deposits Underlying Selected Canals in Central and Western Nebraska: U.S. Geological Survey Fact Sheet 115-03, 6 p., doi:10.3133/fs11503.
LeBlanc, L.R., Panda, S., and Schock, S.G., 1992, Sonar attenuation modeling for classification of marine sediments: The Journal of the Acoustical Society of America, v. 91, p. 116–126, doi: 10.1121/1.402759.
Placzek, Gary, and Haeni, F.P., 1995, Surface-geophysical techniques used to detect existing and infilled scour holes near bridge piers: U.S. Geological Survey Water-Resources Investigations Report 95-4009, 44 p., doi:10.3133/WRI954009.
Sylwester, R.E., 1983, Single channel, high-resolution, seismic-reflection profiling: A review of the fundamentals and instrumentation, in, Geyer, R.A., ed., Handbook of geophysical exploration at sea: Boca Raton, FL, CRC Press, Inc., p. 77-121.
Taylor, D.I., 1992, Nearshore shallow gas around the U.K. coast: Continental Shelf Research, v. 12, no. 10, p. 1135-1144, doi:10.1016/0278-4343(92)90074-T.
Trabant, P.K., Wornardt, W.W., and Vail, P.R., 1997, Seismic Sequence Stratigraphy: Berlin, Springer-Verlag Berlin, 250 p.
Vrbancich, J., Whiteley, R.J., Caffi, P., and Emerson, D.W., 2011, Marine seismic profiling and shallow marine sand resistivity investigations in Broken Bay, NSW, Australia: Exploration Geophysics, v. 42, no. 4, p. 227-238, doi:10.1071/EG11031.