Frequency Domain Electromagnetic (FDEM)
Basic Concept
Frequency domain electromagnetic (FDEM) methods are used to detect variations in subsurface electrical conductivity (or its inverse, resistivity) using electromagnetic induction (EMI) principles. FDEM methods induce alternating current (AC) flow within subsurface electrical conductors and compare the amplitude and phase of the returning signal to those of the transmitted signal. With such signal analysis, FDEM methods can detect the spatial variations of subsurface electrical conductivity, which can be used to estimate material- and hydrogeologic properties.
FDEM methods are noninvasive because, unlike DC resistivity methods, they do not require direct contact with the ground. New FDEM systems allow rapid data collection that may be acquired by only one individual. A variety of equipment is available for used in small- to large scale stationary, mobile, and/or aerial surveys over land or water. Additionally, inversion programs can be used to interpret the data and obtain and map distribution of electrical conductivity with depth (Auken and Christiansen, 2004).
Theory
Frequency domain electromagnetic devices continuously transmit electromagnetic (EM) signal, which is generated using the EM induction (EMI) process. A power source supplies an alternating current (AC) at a specific frequency to a closed, multi-turn coil of wire. Current flow within the transmitter coil generates a magnetic field that surrounds it and penetrates the subsurface. This time-varying primary magnetic field induces an electromotive force in nearby materials due to their EM properties of electrical conductivity, magnetic susceptibility, and dielectric permittivity.
The electromotive force generates an electrical eddy current within subsurface conductors, which produces a secondary magnetic field that is similar to the primary field. Relative to the primary field, the secondary magnetic field has an amplitude and phase shift due to the EM properties of the subsurface materials. An AC is induced within a surface receiver coil as it is energized by the primary and secondary magnetic fields and monitored by a sensor that records the voltage across the coil (Figure 1).
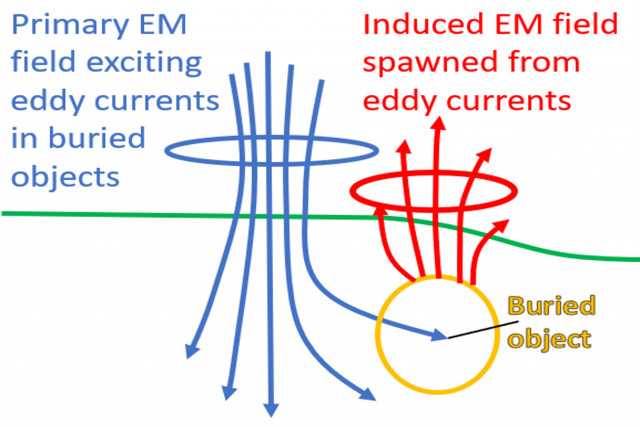
The effects of the primary magnetic field can be counteracted by supplying a current to the receiver that is equal and opposite to that supplied to the transmitter. Thus, the transmitter- and receiver coils are electrically connected, which also allows signal for comparison. Analysis of signal amplitude considers the ratio of the secondary magnetic field to the primary magnetic field. The depth and size of the conductor are the properties most related to and can be estimated using signal amplitude information.
The returning signal can be divided into two parts: the 1) in-phase and 2) quadrature components. The in-phase (or “real”) component has a maximum amplitude that occurs simultaneously with that of the transmitted signal. The quadrature component, which is also called the “out-of-phase” or imaginary component, has a phase shift relative to the transmitted signal. The phase shift of the quadrature component is the fraction of period by which its maximum amplitude lags the maximum amplitude of the transmitted signal.
Though analysis of both components can give information on apparent conductivity and magnetic susceptibility, FDEM methods are primarily used to determine electrical conductivity variations. In general, the returning signal is a complex function of electrical conductivity, operation frequency, and intercoil spacing (i.e., distance between transmitter and receiver coils). However, at low induction numbers, the secondary magnetic field can be described as a simple function of those same parameters, and the quadrature component is proportional to conductivity (McKenna and McKenna, 2010).
Operation under low induction numbers requires an AC frequency low enough so that the electrical skin depth is significantly greater than the intercoil spacing. Skin depth, which is the bottom boundary of the subsurface conductive region through which the majority of current flows, depends on intercoil spacing and operating frequency. Thus, the frequency and/or intercoil spacing can be adjusted to achieve a response that primarily contains the quadrature component, which, thereby simplifies conductivity determination (Wightman and others, 2003).
Applications
The most widely applied FDEM survey method is a deviation of the Slingram method, which involves a transportable transmitter-receiver coil pair. Such tools are constructed with the coils held rigidly in a frame and are typically operated by an individual on foot. However, intercoil spacing is related to depth of investigation (DOI). Thus, certain survey objectives may necessitate the use of an instrument with greater penetration capabilities (e.g., large coils flown above land surface in airborne surveys).
An attractive facet of the modern FDEM methods is their ability to operate using a variety of transmitter and receiver coil orientations. Coil orientation affects the response returned from the subsurface and can be chosen specific to survey objective. FDEM instruments have several of these operational mode options. However, the two most commonly used modes are the horizontal coplanar (i.e., vertical dipole) and vertical coplanar (i.e., horizontal dipole) (Figure 2).

The horizontal coplanar (HCP) orientation has the coils in the same plane oriented parallel to the ground. The HCP operational mode is more sensitive to intercoil spacing and the presence of steeply dipping conductors. The vertical coplanar (VCP) orientation has the coils in a plane that is perpendicular to the ground surface. The VCP mode measures the conductivity of the material surrounding steeply dipping conductors and is less sensitive to intercoil spacing.
The generalized depth of investigation (DOI) of the HCP mode is approximately 1.5 times the intercoil spacing, whereas that of the VCP is typically ¾ of the separation. However, both are affected by the operation frequency and the subsurface electrical conductivity (Von Hebel and others, 2014). Generally, lower frequencies and lower subsurface conductivities allow for greater depths of investigation. After data inversion, a full analysis of DOI can be done using the methods described by Christiansen and Auken (2012).
To determine the vertical variations in conductivity, the intercoil spacing, operating frequency, and/or moment (i.e., current supplied to the transmitter) can be manipulated. If none of these factors are changed, the returning signal represents a nonlinear average of all electrical conductivities within the measurement zone at a point below land surface. Often, variable frequency systems are employed and step through multiple frequencies to collect measurements at a variety of depths at each point along a transect.
The results of an FDEM survey can be plotted as a pseudo-section of apparent conductivity. Though, interpretation accuracy increases if the data are inverted to recover a conductivity model of the earth that matches the measured data (Auken and Christiansen, 2004). If data collection, analysis, and interpretation are executed correctly, the FDEM methods produce images of the subsurface conductivity and have been beneficial in the following applications:
- Mapping soil moisture/porosity/structure
- Mapping soil/groundwater salinity
- Identifying tunnels, unexploded ordinance (UXO), utilities, drums, tanks, etc.
- Mapping groundwater contamination, plume locations, and/or groundwater discharge
- Monitoring changes in fluid conductivity or salt-water intrusion over time
- Mapping bedrock structure and/or permafrost
- General applications (e.g., geologic mapping, mining, archaeology, paleogeography)
Examples/Case studies
Boaga, J., 2017, The use of FDEM in hydrogeophysics: A review: Journal of Applied Geophysics, v. 139, p. 36-46, doi:10.1016/j.jappgeo.2017.02.011.
Abstract: Hydrogeophysics is a rapidly evolving discipline emerging from geophysical methods. Geophysical methods are nowadays able to illustrate not only the fabric and the structure of the underground, but also the subsurface processes that occur within it, as fluids dynamic and biogeochemical reactions. This is a growing wide inter-disciplinary field, specifically dedicated to revealing soil properties and monitoring processes of change due to soil/bio/atmosphere interactions. The discipline involves environmental, hydrological, agricultural research and counts application for several engineering purposes. The most frequently used techniques in the hydrogeophysical framework are the electric and electromagnetic methods because they are highly sensitive to soil physical properties such as texture, salinity, mineralogy, porosity and water content. Non-invasive techniques are applied in a number of problems related to characterization of subsurface hydrology and groundwater dynamic processes. Ground based methods, as electrical tomography, proved to obtain considerable resolution but they are difficult to extend to wider exploration purposes due to their logistical limitation. Methods that don't need electrical contact with soil can be, on the contrary, easily applied to broad areas. Among these methods, a rapidly growing role is played by frequency domain electro-magnetic (FDEM) survey. This is due thanks to the improvement of multi-frequency and multi-coils instrumentation, simple time-lapse repeatability, cheap and accurate topographical referencing, and the emerging development of inversion codes. From raw terrain apparent conductivity meter, FDEM survey is becoming a key tool for 3D soil characterization and dynamics observation in near surface hydrological studies. Dozens of papers are here summarized and presented, in order to describe the promising potential of the technique.
De Smedt, P., Van Meirvenne, M., Saey, T., Baldwin, E., Gaffney, C., and Gaffney, V., 2014, Unveiling the prehistoric landscape at Stonehenge through multi-receiver EMI: Journal of Archaeological Science, v. 50, p. 16-23, doi:10.1016/j.jas.2014.06.020.
Abstract: Archaeological research at Stonehenge (UK) is increasingly aimed at understanding the dynamic of the wider archaeological landscape. Through the application of state-of-the-art geophysical techniques, unprecedented insight is being gathered into the buried archaeological features of the area. However, applied survey techniques have rarely targeted natural soil variation, and the detailed knowledge of the palaeotopography is consequently less complete. In addition, metallic topsoil debris, scattered over different parts of the Stonehenge landscape, often impacts the interpretation of geophysical datasets. The research presented here demonstrates how a single multi-receiver electromagnetic induction (EMI) survey, conducted over a 22 ha area within the Stonehenge landscape, offers detailed insight into natural and anthropogenic soil variation at Stonehenge. The soil variations that were detected through recording the electrical and magnetic soil variability, shed light on the genesis of the landscape, and allow for a better definition of potential palaeoenvironmental and archaeological sampling locations. Based on the multi-layered dataset, a procedure was developed to remove the influence of topsoil metal from the survey data, which enabled a more straightforward identification of the detected archaeology. The results provide a robust basis for further geoarchaeological research, while potential to differentiate between modern soil disturbances and the underlying sub-surface variations can help in solving conservation and management issues. Through expanding this approach over the wider area, we aim at a fuller understanding of the human–landscape interactions that have shaped the Stonehenge landscape.
Delefortrie, S., Hanssens, D., and De Smedt, P., 2018, Low signal-to-noise FDEM in-phase data: Practical potential for magnetic susceptibility modelling: Journal of Applied Geophysics, v. 152, p. 17-25, doi:10.1016/j.jappgeo.2018.03.003.
Abstract: In this paper, we consider the use of land-based frequency-domain electromagnetics (FDEM) for magnetic susceptibility modelling. FDEM data comprises both out-of-phase and in-phase components, which can be related to the electrical conductivity and magnetic susceptibility of the subsurface. Though applying the FDEM method to obtain information on the subsurface conductivity is well established in various domains (e.g. through the low induction number approximation of subsurface apparent conductivity), the potential for susceptibility mapping is often overlooked. Especially given a subsurface with a low magnetite and maghemite content (e.g. most sedimentary environments), it is generally assumed that susceptibility is negligible. Nonetheless, the heterogeneity of the near surface and the impact of anthropogenic disturbances on the soil can cause sufficient variation in susceptibility for it to be detectable in a repeatable way. Unfortunately, it can be challenging to study the potential for susceptibility mapping due to systematic errors, an often poor low signal-to-noise ratio, and the intricacy of correlating in-phase responses with subsurface susceptibility and conductivity. Alongside use of an accurate forward model – accounting for out-of-phase/in-phase coupling – any attempt at relating the in-phase response with subsurface susceptibility requires overcoming instrument-specific limitations that burden the real-world application of FDEM susceptibility mapping. Firstly, the often erratic and drift-sensitive nature of in-phase responses calls for relative data levelling. In addition, a correction for absolute levelling offsets may be equally necessary: ancillary (subsurface) susceptibility data can be used to assess the importance of absolute in-phase calibration though hereby accurate in-situ data is required. To allow assessing the (importance of) in-phase calibration alongside the potential of FDEM data for susceptibility modelling, we consider an experimental test case whereby the in-phase responses of a multi-receiver FDEM instrument are calibrated through downhole susceptibility data. Our results show that, while it is possible to derive approximate susceptibility profiles from FDEM data, robust quantitative analysis hinges on appropriate calibration of the responses.
Doolittle, J.A. and Brevik, E.C., 2014, The use of electromagnetic induction techniques in soils studies: Geoderma, v. 223-225, p. 33-45, doi:10.1016/j.geoderma.2014.01.027.
Abstract: Electromagnetic induction (EMI) has been used to characterize the spatial variability of soil properties since the late 1970s. Initially used to assess soil salinity, the use of EMI in soil studies has expanded to include: mapping soil types; characterizing soil water content and flow patterns; assessing variations in soil texture, compaction, organic matter content, and pH; and determining the depth to subsurface horizons, stratigraphic layers or bedrock, among other uses. In all cases the soil property being investigated must influence soil apparent electrical conductivity (ECa) either directly or indirectly for EMI techniques to be effective. An increasing number and diversity of EMI sensors have been developed in response to users' needs and the availability of allied technologies, which have greatly improved the functionality of these tools. EMI investigations provide several benefits for soil studies. The large amount of georeferenced data that can be rapidly and inexpensively collected with EMI provides more complete characterization of the spatial variations in soil properties than traditional sampling techniques. In addition, compared to traditional soil survey methods, EMI can more effectively characterize diffuse soil boundaries and identify areas of dissimilar soils within mapped soil units, giving soil scientists greater confidence when collecting spatial soil information. EMI techniques do have limitations; results are site-specific and can vary depending on the complex interactions among multiple and variable soil properties. Despite this, EMI techniques are increasingly being used to investigate the spatial variability of soil properties at field and landscape scales.
McKenna, S.P. and McKenna, J.R., 2010, Modeling and analysis of the response of a triaxial, frequency-domain electromagnetic induction sensor to a buried linear conductor: Geophysics, v. 75, no. 1, p. 1JF-Z16, doi:10.1190/1.3267876.
Abstract: This paper presents analytical modeling results for a triaxial frequency-domain electromagnetic-induction (EMI) sensor over a homogeneous earth containing a long linear conductor. Although the conductor studied is intended to represent an underground wire or pipe, it can represent any subsurface, linear geologic structure that can channel current. Treating the sensor transmitter as a vertical magnetic dipole, the model combines the well-known solution for the magnetic field arising from the interaction with the earth with the solution for the induced magnetic field from the excited subsurface conductor. Expressions for the three components of the magnetic field at an arbitrary point above the earth are presented. Two types of coupled, moving transmitter-receiver configurations (coaxial and coplanar) were considered, and the model is sufficiently flexible to allow for many other sensor variations to be studied. Characteristics of the sensor signals were explored through several parametric modeling studies that demonstrate the sensitivity of the signals to transmitter frequency, earth conductivity, conductor depth, sensor geometry, and crossing angle. Using simple relationships developed from analysis of the sensor signals, key parameters such as conductor depth and orientation can be estimated. The ability of the model to predict and characterize sensor output should prove helpful in distinguishing between geologic features and man-made underground infrastructure. These modeling results also are expected to facilitate frequency-domain EMI data analysis and interpretation, sensor design and operation, and the development of detection and classification algorithms.
Saey, T., Van Meirvenne, M., Dewilde, M., Wyffels, F., De Smedt, P., Meerschman, E., Islam, M.M., Meeuws, F., and Cockx, L., 2010, Combining multiple signals of an electromagnetic induction sensor to prospect land for metal objects: Near Surface Geophysics, v. 9, no. 4, p. 309-318, doi:10.3997/1873-0604.2010070.
Abstract: Buried unexploded ammunition is a major problem on arable land in former battle areas. Many battlefields of the First World War (WWI) still contain a lot of unexploded shells just below the plough layer, posing serious threats to soil editors and trenchers. Electromagnetic induction (EMI) sensors have been used for a variety of agricultural and archaeological purposes to map the natural soil variability and to locate buried archaeological remains. Besides its sensitivity to variations in soil texture and anthropogenic disturbances, EMI proves to respond strongly to metal objects in the soil. Most EMI sensors rely on a single signal, with magnitude and sign of the metal anomalies differing according to the instruments coil distance and separation. The multi‐coil EMI sensor, the DUALEM‐21S, provides four simultaneous apparent electrical conductivity (ECa) signals enhancing significantly the possibilities for signal processing. To calibrate our instrument, we buried different masses of metal at different depths. The four ECa measurements showed a response to the metal objects down to 1.2 m. The measurements were subtracted by their gradual trend to obtain the local anomalies (ΔECa). A combination of these four ΔECa’s was used to amplify the signal response to metal, influenced by both depth and mass of the buried objects. At an intensively shelled former WWI battle field near Ypres (Belgium), a detailed prospection was conducted with the DUALEM‐21S. Based on our multi‐signal procedure, we located 40 positions, 20 where we predicted buried metal and 20 where we expected that no metal was present within 1.2 m depth. There were no false negative predictions and at the 20 locations where we expected metal, shells up to 90 kg were excavated. As a final outcome we produced a map with predictions of the mass of metal objects in the soil assuming a fixed depth and alternatively a map with predictions of the depth of metal objects assuming a given mass. Apart from their potential for agricultural and archaeological investigations, multi‐ECa signals were shown to be useful for locating metal objects, like unexploded WWI shells, in the top 1.2 m of soil.
Sheets, K.R. and Hendrickx, J.M.H., 1995, Noninvasive Soil Water Content Measurement Using Electromagnetic Induction: Water Resources Research, v. 31, no. 10, p. 2401-2409, doi:10.1029/95WR01949.
Abstract: The feasibility of soil water content measurement using electromagnetic induction was investigated in an arid region of southern New Mexico. Soil water measurements were taken monthly with a neutron probe at 65 equally spaced stations along a 1950‐m transect. At the same time, noninvasive electrical conductivity measurements of the soil were taken with a Geonics EM‐31 ground conductivity meter. Using 16 months of measurements, we found a linear relationship exists between bulk soil electrical conductivity and total soil water content in the top 1.5 m of the profile. A simple linear regression model was developed to describe the relationship between soil water content and bulk soil electrical conductivity. The spatial and temporal accuracy of the regression model is addressed as well as the total number of neutron access tubes needed to accurately calibrate the model. By comparison with the neutron scattering method the electromagnetic induction method is quite accurate for the prediction of water content changes over time. The speed and ease of use combined with the accuracy of the measurements make the ground conductivity meter a valuable tool for rapid, noninvasive soil water measurements.
Verhegge, J., Saey, T., Van Meirvenne, M., Missiaen, T., and Crombé, P., 2017, Reconstructing Early Neolithic Paleogeography: EMI‐Based Subsurface Modeling and Chronological Modeling of Holocene Peat below the Lower Scheldt Floodplain in NW Belgium: Geoarchaeology, v. 32, no. 2, p. 159-176, doi:10.1002/gea.21581.
Abstract: Well‐preserved prehistoric landscapes and sites have been found, buried deeply below the Holocene peat or floodplain deposits of “Waasland Scheldt polders.” During the mid‐to‐late Holocene, Late Weichselian (river) dunes within the floodplain and river flanks were favored locations for Final Early Neolithic occupation. Available living space was determined by the dune topography and elevation of the peat at that time. Therefore, an elevation model of the peat base was created using multireceiver electromagnetic induction (EMI) survey data. Electrical conductivity data of a dune were collected and 1D inverted within a three‐layered soil model with variable electrical conductivity of the top layer and variable depth to the base of the middle layer (i.e., the peat). The modeled peat base depth was calibrated and validated, and eventually replaced by depth data from coring and cone penetration measurements wherever depth modeling from inverting the EMI measurements proved inaccurate. Using the resulting peat base elevation model, a paleogeographic map at the time of the modeled end date of Mesolithic–Neolithic transitional Swifterbant Culture sites nearby was created by chronologically modeling the peat elevation at that time. The developed paleogeographic mapping methodology can be used for subsequent archaeological prospection by core sampling or to contextualize excavated sites.
Von Hebel, C., Rudolph, S., Mester, A., Huisman, J.A., Kumbhar, P., Vereecken, H., and van der Kruk, J., 2014, Three‐dimensional imaging of subsurface structural patterns using quantitative large‐scale multiconfiguration electromagnetic induction data: Water Resources Research, v. 50, p. 2732-2748,doi: 10.1002/2013WR014864.
Abstract: Electromagnetic induction (EMI) systems measure the soil apparent electrical conductivity (ECa), which is related to the soil water content, texture, and salinity changes. Large‐scale EMI measurements often show relevant areal ECa patterns, but only few researchers have attempted to resolve vertical changes in electrical conductivity that in principle can be obtained using multiconfiguration EMI devices. In this work, we show that EMI measurements can be used to determine the lateral and vertical distribution of the electrical conductivity at the field scale and beyond. Processed ECa data for six coil configurations measured at the Selhausen (Germany) test site were calibrated using inverted electrical resistivity tomography (ERT) data from a short transect with a high ECa range, and regridded using a nearest neighbor interpolation. The quantitative ECa data at each grid node were inverted using a novel three‐layer inversion that uses the shuffled complex evolution (SCE) optimization and a Maxwell‐based electromagnetic forward model. The obtained 1‐D results were stitched together to form a 3‐D subsurface electrical conductivity model that showed smoothly varying electrical conductivities and layer thicknesses, indicating the stability of the inversion. The obtained electrical conductivity distributions were validated with low‐resolution grain size distribution maps and two 120 m long ERT transects that confirmed the obtained lateral and vertical large‐scale electrical conductivity patterns. Observed differences in the EMI and ERT inversion results were attributed to differences in soil water content between acquisition days. These findings indicate that EMI inversions can be used to infer hydrologically active layers.
References
Auken, E., and Christiansen, A.V., 2004, Layered and laterally constrained 2D inversion of resistivity data: Geophysics, v. 69, no. 3, p. 752-761, doi:10.1190/1.1759461.
Boaga, J., 2017, The use of FDEM in hydrogeophysics: A review: Journal of Applied Geophysics, v. 139, p. 36-46, doi:10.1016/j.jappgeo.2017.02.011.
De Smedt, P., Van Meirvenne, M., Saey, T., Baldwin, E., Gaffney, C., and Gaffney, V., 2014, Unveiling the prehistoric landscape at Stonehenge through multi-receiver EMI: Journal of Archaeological Science, v. 50, p. 16-23, doi:10.1016/j.jas.2014.06.020.
Delefortrie, S., Hanssens, D., and De Smedt, P., 2018, Low signal-to-noise FDEM in-phase data: Practical potential for magnetic susceptibility modelling: Journal of Applied Geophysics, v. 152, p. 17-25, doi:10.1016/j.jappgeo.2018.03.003.
Doolittle, J.A. and Brevik, E.C., 2014, The use of electromagnetic induction techniques in soils studies: Geoderma, v. 223-225, p. 33-45, doi:10.1016/j.geoderma.2014.01.027.
McKenna, S.P. and McKenna, J.R., 2010, Modeling and analysis of the response of a triaxial, frequency-domain electromagnetic induction sensor to a buried linear conductor: Geophysics, v. 75, no. 1, p. 1JF-Z16, doi:10.1190/1.3267876.
Mussett, A.E. and Khan, M.A., 2000, Electromagnetic Methods, in Looking into The Earth: An Introduction to Geological Geophysics: New York, Cambridge University Press, p 210-231.
Saey, T., Van Meirvenne, M., Dewilde, M., Wyffels, F., De Smedt, P., Meerschman, E., Islam, M.M., Meeuws, F., and Cockx, L., 2010, Combining multiple signals of an electromagnetic induction sensor to prospect land for metal objects: Near Surface Geophysics, v. 9, no. 4, p. 309-318, doi:10.3997/1873-0604.2010070.
Sheets, K.R. and Hendrickx, J.M.H., 1995, Noninvasive Soil Water Content Measurement Using Electromagnetic Induction: Water Resources Research, v. 31, no. 10, p. 2401-2409, doi:10.1029/95WR01949.
Verhegge, J., Saey, T., Van Meirvenne, M., Missiaen, T., and Crombé, P., 2017, Reconstructing Early Neolithic Paleogeography: EMI‐Based Subsurface Modeling and Chronological Modeling of Holocene Peat below the Lower Scheldt Floodplain in NW Belgium: Geoarchaeology, v. 32, no. 2, p. 159-176, doi:10.1002/gea.21581.
Von Hebel, C., Rudolph, S., Mester, A., Huisman, J.A., Kumbhar, P., Vereecken, H., and van der Kruk, J., 2014, Three‐dimensional imaging of subsurface structural patterns using quantitative large‐scale multiconfiguration electromagnetic induction data: Water Resources Research, v. 50, p. 2732-2748, doi: 10.1002/2013WR014864.
Wightman, W.E., Jalinoos, F., Sirles, P., and Hanna, K., 2003, Application of Geophysical Methods to Highway Related Problems: Lakewood, CO, Federal Highway Administration, Office of Bridge Technology, 742 p.