Waterborne Ground Penetrating Radar
Basic Concept
Ground penetrating radar (GPR) is an electromagnetic (EM) method that transmits radio-wave pulses into earth materials to image the subsurface. GPR capitalizes on the effects that the EM-material properties of dielectric permittivity, electrical conductivity, and magnetic permeability have on EM-energy propagation and reflection. A portion of the radar-wave energy that encounters a subsurface interface with sufficiently different EM properties can be reflected. The remaining energy will continue to propagate until it is reflected again or dissipates completely.
Returning radar signals are recorded at the GPR receiver, and the amplitude variations and wave velocity-dependent travel times are measured (see Figure 1 below). GPR data can be plotted in cross section in real-time, depict the subsurface electrical structure, and used to determine the depth, geometry, and characteristics of radar-reflecting subsurface features. (Beres and Haeni, 1994)
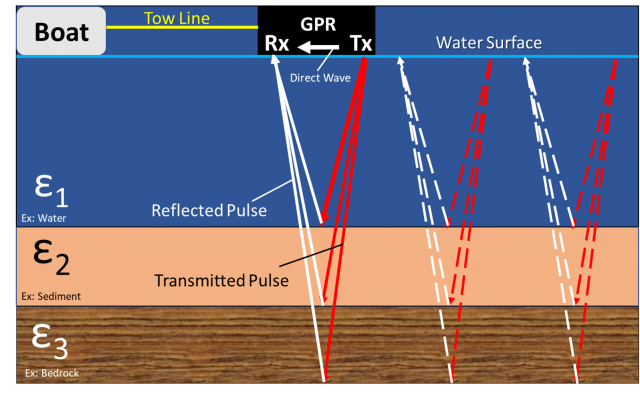
While the development and primary application of GPR has been land-based, GPR is also commonly used in marine applications. Waterborne GPR surveys can be conducted by towing a GPR antenna from a non-electrically conductive vessel (see Figure 2 below) along or submerged within a water body (e.g. Sauck and Seng, 1994). In addition to the land-based applications of GPR, waterborne GPR surveys are well-suited for applications such as determining water depth, sub bottom-sediment thickness, and surface-water/groundwater connection.
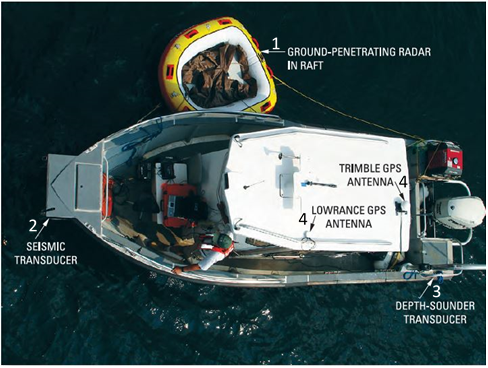
Theory
The GPR method relies on the principles of electromagnetic theory. Waterborne GPR uses high-frequency (typically 100-2,000 MHz) pulsed-radio waves that are generated by a transmitter antenna. The portion of the transmitted-wave field that propagates through the water and into the subsurface is the radar (i.e., Radio Detection And Ranging) signal used to image the subsurface. The receiver antenna measures the strength (i.e., amplitude) of the reflections off subsurface features and time required to detect them.
Radar propagation into a surface water body and underlying sediment is impacted by contrasts in the electromagnetic properties of the earth materials (Daniels, 1989). Radar waves reflect off subsurface interfaces with sufficient contrast in wave impedance, which is a material property related to dielectric permittivity, electrical conductivity, and magnetic permeability. The reflected-signal amplitude depends on the impedance-contrast magnitude, and remaining wave energy can continue traveling through the subsurface until it reaches another reflector or attenuates completely.
While wave impedance can be described as a relation between the three above-mentioned electromagnetic material, electrical conductivity does not significantly influence reflection. Furthermore, the magnetic permeability is often not high enough to impact the interpretation of GPR data and can be considered negligible. However, under certain conditions (e.g., in the presence of magnetite), magnetic permeability can strongly influence radar attenuation and velocity, and such effects can be considered (Van Dam and others, 2013).
Thus, in most subsurface environments, the contrast in dielectric permittivity (ε) is the primary factor that controls GPR signal return (see Figure 1 above). Dielectric permittivity is the capacity of a material to polarize under the influence of an electric field. Note that relative permittivity (εR) can describe dielectric properties and has a value relative to the permittivity of a vacuum. At the frequencies used by GPR, dielectric permittivity is strongly sensitive to variations in water content.
The GPR method uses the two-way travel time (TWT) and velocity (v) of the wave to estimate the depths of reflectors below a reference point. Through space, radar waves travel at the speed of light, and their velocity decreases with increasing dielectric permittivity. However, GPR analysis typically uses an average velocity for each layer or medium. Average values can be estimated using a reference reflector at a known depth, the common-midpoint survey method (land-based GPR), or literature values (Musset and Khan, 2000).
Applications
Waterborne GPR surveys typically employ the common-offset survey configuration where an antenna pair (i.e., a bistatic antenna) is mounted on towed raft. The GPR unit is moved along a transect while the system transmits radar pulses that last a few nanoseconds and are within the frequency range of 100 to 2,000 MHz. The returning signal amplitude is measured over time, and signal traces are displayed live on a digital handheld recorder and stored for analysis.
One of the most attractive assets of the GPR method is its high-resolution imaging capabilities, which aid the identification of key structural or hydrogeological subsurface features. Waterborne GPR data can produce high-resolution, two-dimensional, cross-sectional images of sub-bottom structures at shallow depths in estuarine, riverine, and lacustrine environments. Figure 3 shows an example of a waterborne GPR profile and structural interpretations.
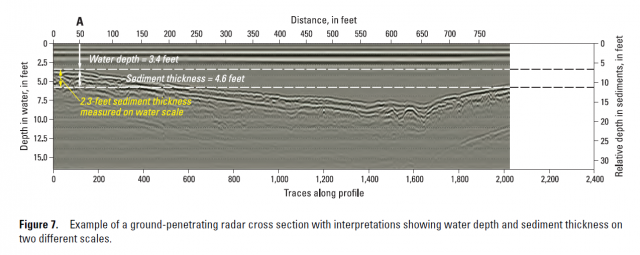
However, there is a tradeoff between depth of signal penetration and data resolution, which improves with increased signal frequency. This is due to the relation between attenuation and signal frequency (i.e., lower frequencies attenuate less and penetrate deeper than higher frequencies and with lower resolution). Thus, an optimal operating frequency should be determined prior to field work based on site parameters and survey objectives.
Additionally, radar waves attenuate (i.e., lose amplitude) as they propagate through and interact with materials. Radar energy is lost through the antennas themselves, surface coupling, geometric-signal spreading, scattering, and intrinsic attenuation. Intrinsic attenuation (α) is the loss of radar energy through absorption and greatly affects the depth of investigation (DOI).
Radar waves travel further in fresher waters than in conductive waters. In practice, water-column conductivities below 200 microSiemens per centimeter allow signal penetration into sub-bottom sediments up to around 25 meters. GPR is not effective in highly conductive water (e.g., saline water bodies), as the signal is highly attenuated by electrically conductive media.
Though the GPR record may reveal very detailed sub-horizontal stratigraphy, there is no absolute correlation between reflections and geologic or hydrologic structures or properties (Wightman and others, 2003). Additionally, radar waves are conical and reflections off discrete objects or point reflectors (e.g., pipe/utilities, vertical/dipping structures) appear in the GPR record as hyperbolic. However, with adequate velocity information, a data migration (i.e., processing) can be performed that will focus discrete reflectors and correct the slope of dipping reflectors.
Like other EM methods, waterborne GPR surveys may experience possible interference from radio-frequency transmitters and large metal structures (e.g. power lines, cars). Additionally, the depth, geometry (i.e., size, orientation) and composition of the target may not preclude it from being detected by the GPR method. Despite some pitfalls, the waterborne GPR method has been successfully used as an investigative tool applied to hydrogeological, environmental, engineering, and archaeological studies to aid the following:
- Mapping of bedrock configuration and depth
- Locating of pipes, tanks, and other utilities
- Determination of water depth
- Assessing of streambed conductance and
- Determination of sub-bottom sediment thickness
- Assessing likelihood of surface-water/groundwater connections
- Mapping of fractures, changes of rock type, and rock fabrics
- Locating of fresh water/saline water interface.
Examples/Case studies
Aksu, H.H., Kanbur, M.Z., Görmüş, M., 2017, Investigation of the Kumdanlı and surrounding faults on the Eğirdir Lake by conducting ground penetrating radar (GPR) profiles: Arabian Journal of Geosciences, v. 10, doi:10.1007/s12517-017-3162-2.
Abstract: A ground penetrating radar (GPR) survey was performed on the Eğirdir Lake to obtain the structural properties of Kumdanlı and surrounding faults. GPR data was collected along ten profiles in various directions by using 100–200-MHz GPR antennas. The radar sections showed that the Kumdanlı Fault, SW-NE in direction, and also some other neighboring NW-SE normal faults have played an active role in forming the Eğirdir-Hoyran Lakes. The deformation and the geometry obtained from the results show that the Kumdanlı Fault is a sinistral strike-slip fault including oblique and segmented displacements. The other faults (Kemerdamı and Akkeçili) have mainly normal fault systems. Their trends are generally NW-SE in direction. It is also observed that they are younger than the Kumdanlı Fault system. These fault properties demonstrate the Eğirdir Lake side has an extensional structure trending SW-NE.
Banks, W.S.L., and Johnson, C.D., 2011, Collection, processing, and interpretation of ground-penetrating radar data to determine sediment thickness at selected locations in Deep Creek Lake, Garrett County, Maryland, 2007: U.S. Geological Survey Scientific Investigations Report 2011–5223, 36 p., doi:10.3133/sir20115223.
Abstract: The U.S. Geological Survey collected geophysical data in Deep Creek Lake in Garrett County, Maryland, between September 17 through October 4, 2007 to assist the Maryland Department of Natural Resources to better manage resources of the Lake. The objectives of the geophysical surveys were to provide estimates of sediment thickness in shallow areas around the Lake and to test the usefulness of three geophysical methods in this setting. Ground-penetrating radar (GPR), continuous seismic-reflection profiling (CSP), and continuous resistivity profiling (CRP) were attempted. Nearly 90 miles of GPR radar data and over 70 miles of CSP data were collected throughout the study area. During field deployment and testing, CRP was determined not to be practical and was not used on a large scale. Sediment accumulation generally could be observed in the radar profiles in the shallow coves. In some seismic profiles, a thin layer of sediment could be observed at the water bottom. The radar profiles appeared to be better than the seismic profiles for the determination of sediment thickness. Although only selected data profiles were processed, all data were archived for future interpretation.
This investigation focused on selected regions of the study area, particularly in the coves where sediment accumulations were presumed to be thickest. GPR was the most useful tool for interpreting sediment thickness, especially in these shallow coves. The radar profiles were interpreted for two surfaces of interest—the water bottom, which was defined as the “2007 horizon,” and the interface between Lake sediments and the original Lake bottom, which was defined as the “1925 horizon”—corresponding to the year the Lake was impounded. The ground-penetrating radar data were interpreted on the basis of characteristics of the reflectors. The sediments that had accumulated in the impounded Lake were characterized by laminated, parallel reflections, whereas the subsurface below the original Lake bottom was characterized by more discontinuous and chaotic reflections, often with diffractions indicating cobbles or boulders. The reflectors were picked manually along the water bottom and along the interface between the Lake sediments and the pre-Lake sediments. A simple graphic approach was used to convert travel times to depth through water and depth through saturated sediments using velocities of the soundwaves through the water and the saturated sediments. Nineteen cross sections were processed and interpreted in 9 coves around Deep Creek Lake, and the difference between the 2007 horizon and the 1925 horizon was examined. In most areas, GPR data indicate a layer of sediment between 1 and 7 feet thick. When multiple cross sections from a single cove were compared, the cross sections indicated that sediment thickness decreased toward the center of the Lake.
Beres Jr., M., and Haeni, F.P., 1991, Application of Ground-Penetrating-Radar Methods in Hydrologic Studies: Groundwater, v. 29, no. 3, p. 375-386, doi:10.1111/j.1745-6584.1991.tb00528.x.
Abstract: A ground‐penetrating‐radar system was used to study selected stratified‐drift deposits in Connecticut. Ground‐penetrating radar is a surface‐geophysical method that depends on the emission, transmission, reflection, and reception of an electromagnetic pulse and can produce continuous high‐resolution profiles of the subsurface rapidly and efficiently. Traverse locations on land included a well field in the town of Mansfield, a sand and gravel pit and a farm overlying a potential aquifer in the town of Coventry, and Haddam Meadows State Park in the town of Haddam. Traverse locations on water included the Willimantic River in Coventry and Mansfield Hollow Lake in Mansfield. The penetration depth of the radar signal ranged from about 20 feet in fine‐grained glaciolacustrine sediments to about 70 feet in coarse sand and gravel. Some land records in coarse‐grained sediments show a distinct, continuous reflection from the water table about 5 to 11 feet below land surface. Parallel reflectors on the records are interpreted as fine‐grained sediments. Hummocky or chaotic reflectors are interpreted as cross‐bedded or coarse‐grained sediments. Other features observed on some of the radar records include the till and bedrock surface. Records collected on water had distinct water‐bottom multiples (more than one reflection) and diffraction patterns from boulders. The interpretation of the radar records, which required little or no processing, was verified by using lithologie logs from test holes located along some of the land traverses and near the water traverses.
Bradbury, K.R., and Taylor, R.W., 1984, Determination of the Hydrogeologic Properties of Lakebeds Using Offshore Geophysical Surveys: Groundwater, v. 22, no. 6, p. 690-695, doi:10.1111/j.1745-6584.1984.tb01437.x.
Abstract: Studies of the relationships between ground‐water systems and surface‐water systems (lakes) generally require knowledge of the hydrogeologic properties of lakebed materials. Direct measurement of these properties may be prohibitively expensive or difficult in an offshore environment. A correlation between longitudinal conductance, a geoelectric parameter, and lakebed leakance (vertical hydraulic conductivity divided by thickness) may provide a rapid and inexpensive method for estimating lakebed hydrogeological properties using data collected by offshore seismic and electrical surveys. A test of the method at three study sites in Lake Michigan shows a positive linear relationship between the logarithm of longitudinal conductance and the logarithm of lakebed leakance at the three sites.
Buynevich, I.V., and Fitzgerald, D., 2003: High-Resolution Subsurface (GPR) Imaging and Sedimentology of Coastal Ponds, Maine, U.S.A.: Implications for Holocene Back-Barrier Evolution: Journal of Sedimentary Research, v. 73, no. 4, 559-571, doi:10.1306/121802730559.
Abstract: Ground-penetrating radar (GPR) transects and sediment cores have been used to examine the basement morphology, stratigraphy, and environmental history of maritime ponds along the pen-insular coast of Maine. Silver Lake, Lily Pond, and North Pond are shallow (3 m) water bodies bordered by steep bedrock ridges in the north, east, and west, and sandy barriers to the south. The bedrock basins of the ponds are formed in metasedimentary rocks surrounded by resistant pegmatitic intrusions. A dense network of GPR traverses obtained over the ice-covered Silver Lake reveals a series of prominent wavy-parallel and basin-fill reflector geometries terminating against the bedrock or grading into the barrier sediments and interpreted as organic lake-bottom facies. The transparent units represent sand-rich horizons, mostly eolian in origin. Convex-up structures found both on the surface and within the basin-fill sequence are interpreted as pre-served parts of coastal dunes. The present study indicates that fresh-water conditions prevailed since at least 4.6 ka, with an initial sedimentation rate of 1.7 mm/yr. The position of this unit below the con-temporary sea level suggests presence of a welded barrier by that time. Radar profiles taken along the shores of Lily Pond, a small water body behind the Sand Dune Barrier, indicate a significantly larger areal extent of the pond in the past. A succession of organic deposits over-lying a Pleistocene glaciomarine unit indicates progressive inundation of the paleo-lagoon by rising sea level. Saltwater peat seaward of Lily Pond was buried by washover sands about 1.2 ka, and a narrow pond existed here prior to dredging and artificial infilling of its eastern part in the 1950s–60s. The organic and eolian units are absent in the North Pond, where sedimentary fill consists of glaciomarine clay overlain by marine sands. A proposed three-stage model of pond evolution along an embayed coastline consists of: (1) organic accumulation in an up-land depression during lower sea level; (2) predominantly washover or tidal deposition in a lagoon (Stage 2a) or blocked coastal pond (Stage 2b) during initial transgression, and (3) mainly eolian and organic de-position behind a prograded or aggraded barrier. Future accelerated rise in relative sea level and inadequate sediment supply will cause many back-barrier ponds to reenter Stage 2 of the proposed model.
Haeni, F.P., 1996, Use of ground-penetrating radar and continuous seismic reflection profiling on surface-water bodies in environmental and engineering studies: Journal of Environmental and Engineering Geophysics, v. 1, no. 1, p. 27-35, doi:10.4133/JEEG1.1.27.
Abstract: Ground-penetrating radar (GPR) and continuous seismic-reflection profiling (CSP) on shallow rivers, lakes, and ponds are efficient and economical ways of obtaining subsurface hydrologic and geologic information for environmental and engineering studies. These methods are similar in that they produce continuous subsurface profiles, are easy to use in some applications, and the records can occasionally be straightforward to interpret. They are dissimilar in that GPR cannot penetrate electrically conductive water or subsurface sediments and CSP usually cannot operate I n water less than 5 feet (ft.) deep.
GPR records collected on a lake in New Hampshire have been interpreted to estimate the depth to bedrock and to evaluate the grain-size characteristics of the underlying stratified drift at the lakeshore boundary. In a pond in Massachusetts CSP and GPR were used to determine depth to bedrock and the grain-size characteristics of the sub-bottom materials in part of the pond. Water-column multiple reflections, depth and conductivity of water and subsurface materials, and diffractions degraded the quality of the GPR records. CSP records collected in the Connecticut River near Hartford, Connecticut were used to estimate the depth of till and bedrock interfaces and to evaluate grain-size characteristics of subsurface materials. Interpreted CSP records also can indicate bedding planes within consolidated rock units. Water column multiple reflections and very shallow water degraded the quality of the CSP records.
GPR and CSP methods have been used to delineate infilled scour holes near bridge piers. Scour holes that were filled with up to 8 ft. of loose sand were mapped during engineering scour studies near a bridge in Connecticut.
Because GPR and CSP operate on different physical principles, the two geophysical methods complement each other. Depending on the required depth of penetration and the degree of resolution needed, one or both of these methods can be used to acquire accurate and reliable subsurface hydrologic and geologic information critical to environmental and engineering studies.
Powers, C.J., Haeni, F.P., and Smith, S., 1999, Integrated use of continuous seismic-reflection profiling and ground-penetrating radar methods at John’s Pond, Cape Cod, Massachusetts, in Proceedings, Symposium on the Application of Geophysics to Engineering and Environmental Problems: Oakland, CA, Environmental and Engineering Geophysical Society, p. 359-368.
Abstract: Continuous seismic-reflection profiling (CSP) and ground-penetrating radar (GPR) surveys were conducted by the U.S. Geological Survey in April 1998 over the northern part of John’s Pond, a glacial kettle pond southeast of Otis Air National Guard Base, Cape Cod, Massachusetts. The surveys were conducted to delineate the types and thickness of sedimentary units that may control the infiltration of contaminated groundwater into John’s Pond.
Sand-and-gravel deposits, collapse features and recent organic sediments were imaged with the CSP and GPR methods. Hummocky to chaotic reflections were interpreted as sand and-gravel deposits. Slightly wavy, parallel reflections located in depressions in the sand-and gravel deposits were interpreted as filled collapse features. Lower amplitude, horizontal, laminar reflections were interpreted as organic sediments. Entrapped methane gas within some of the organic sediments created a reflection zone that obscured deeper reflections in the CSP records.
The CSP and GPR methods provide complementary information over most of the surveyed part of the pond. The methods detect similar interfaces, but a particular interface may produce a stronger reflection in one record than in the other. For example, regions of the pond containing organic sediments with entrapped methane gas, which prevent penetration of the acoustic signal, were penetrated and imaged by GPR. Conversely, regions of the pond containing electrically conductive sediments or deep water, which attenuate the GPR signal, were imaged using CSP. The CSP and GPR data were interpreted to generate a bathymetric map and a map of sediment type and thickness beneath John’s Pond.
Sambuelli, L., Calzoni, C., and Pesenti, M., 2009, Waterborne GPR survey for estimating bottom-sediment variability: A survey on the Po River, Turin, Italy: Geophysics, v. 74, no. 4, p. 1JA-Z73, doi:10.1190/1.3119262.
Abstract: We conducted an integrated geophysical survey on a stretch of the river Po in order to check the GPR ability to discriminate the variability of riverbed sediments through an analysis of the bottom reflection amplitudes. We conducted continuous profiles with a 200-MHzGPR system and a handheld broadband EM sensor. A conductivity meter and a TDR provided punctual measurements of water conductivity, permittivity, and temperature. The processing and interpretation of the GEM-2 and GPR data were enhanced by reciprocal results and by integration with the punctual measurements of the EM properties of the water. We used a processing flow that improved the radargram images and preserved the amplitude ratios among the different profiles and the frequency content at the bottom reflection signal. We derived the water attenuation coefficient both from the punctual measurements using the Maxwell formulas and from the interpretation of the GPR data, finding an optimal matching between the two values. The GPR measurements provided maps of the bathymetry and of the bottom reflection amplitude. The high reflectivity of the riverbed, derived from the GPR interpretation, agreed with the results of the direct sampling campaign that followed the geophysical survey. The variability of the bottom-reflection-amplitudes map, which was not confirmed by the direct sampling, could also have been caused by scattering phenomena due to the riverbed clasts which are dimensionally comparable to the wavelength of the radar pulse.
Sambuelli, L., and Bava, S., 2012, Case study: A GPR survey on a morainic lake in northern Italy for bathymetry, water volume and sediment characterization: Journal of Applied Geophysics, v. 81, p. 48-56, doi:10.1016/j.jappgeo.2011.09.016.
Abstract: We carried out an extensive waterborne GPR survey consisting of 50 profiles with a total length of nearly 37 km on the morainic lake of Candia northerly Turin (Italy). Our aim was to test the capability of GPR to estimate the bathymetry, the water volume and the sediment type. We enhanced and controlled the GPR data processing and interpretation with bathymetry acquired with an acoustic echo sounder and measured conductivity and temperature profile of the water column with a multiparametric probe. We also analyzed the diffraction hyperbola that originated within the sediments in order to estimate the velocity and relative permittivity. With the permittivity and dielectric mixing rules, we estimated the porosity of the sediments above the diffracting objects and drew a map of the bottom lake porosity.
Sauck, W.A., and Seng, D.L., 1994, Near-shore sand thickness and stratigraphy mapping with a submerged GPR antenna system; Southeast Lake Michigan (Abstract): Geological Society of America Abstracts with Programs, v. 26, no. 5, p. 59.
Abstract: Twenty-one shore perpendicular profiles, spaced at nominal 5 km intervals, have been surveyed with a bottom-sled mounted Ground Penetrating Radar (GPR) antenna system between Benton Harbor, MI, and Gary, IN. Either a commercial 500 MHz or a custom 145 MHz antenna were used. The bottom sled also carried an upward looking SONAR transducer to give concurrent water depth, and was towed from the beach out to water depths of 6 meters or more, usually ending about 500 meters from shore. Bedding structures and details are clearly visible on the GPR sections within the sand bars and sand blankets. Bottom morphology and the nature of the sand bodies change markedly from the NE to the SW limits of the survey area. At the NE profiles there are multiple, pronounced (or high amplitude) offshore bars, with the substrate (glacial clay, shale, or silty sand) exposed or nearly exposed between bars. Internal structure is generally foreset or cross bedding in the bars. Sand was thin or missing immediately to the Sw of several other jetty structures in addition to the one at St. Joseph. In general the sand bars became much less pronounced to the SW, and internal structures were dominated by parallel bedding and subtle angular unconformities. Near St. Joseph, the exposed substrate is almost certainly being eroded, even to water depths as great as 6 meters. Thus, the equilibrium bottom profile continues to deepen shoreward, causing the continued threat of bluff erosion in spite of annual beach nourishment efforts at this site
References
Aksu, H.H., Kanbur, M.Z., Görmüş, M., 2017, Investigation of the Kumdanlı and surrounding faults on the Eğirdir Lake by conducting ground penetrating radar (GPR) profiles: Arabian Journal of Geosciences, v. 10, doi:10.1007/s12517-017-3162-2.
Banks, W.S.L., and Johnson, C.D., 2011, Collection, processing, and interpretation of ground-penetrating radar data to determine sediment thickness at selected locations in Deep Creek Lake, Garrett County, Maryland, 2007: U.S. Geological Survey Scientific Investigations Report 2011–5223, 36 p., doi:10.3133/sir20115223.
Beres Jr., M., and Haeni, F.P., 1991, Application of Ground-Penetrating-Radar Methods in Hydrologic Studies: Groundwater, v. 29, no. 3, p. 375-386, doi:10.1111/j.1745-6584.1991.tb00528.x.
Bradbury, K.R., and Taylor, R.W., 1984, Determination of the Hydrogeologic Properties of Lakebeds Using Offshore Geophysical Surveys: Groundwater, v. 22, no. 6, p. 690-695, doi:10.1111/j.1745-6584.1984.tb01437.x.
Buynevich, I.V., and Fitzgerald, D., 2003: High-Resolution Subsurface (GPR) Imaging and Sedimentology of Coastal Ponds, Maine, U.S.A.: Implications for Holocene Back-Barrier Evolution: Journal of Sedimentary Research, v. 73, no. 4, 559-571, doi:10.1306/121802730559.
Daniels, J.J., 1989, Fundamentals of ground penetrating radar, in Proceedings, Symposium on the Application of Geophysics to Engineering and Environmental Problems: Golden, CO, Environmental and Engineering Geophysical Society, p. 62-142, doi:10.4133/1.2921864.
Haeni, F.P., 1996, Use of ground-penetrating radar and continuous seismic reflection profiling on surface-water bodies in environmental and engineering studies: Journal of Environmental and Engineering Geophysics, v. 1, no. 1, p. 27-35, doi:10.4133/JEEG1.1.27.
Powers, C.J., Haeni, F.P., and Smith, S., 1999, Integrated use of continuous seismic-reflection profiling and ground-penetrating radar methods at John’s Pond, Cape Cod, Massachusetts, in Proceedings, Symposium on the Application of Geophysics to Engineering and Environmental Problems: Oakland, CA, Environmental and Engineering Geophysical Society, p. 359-368.
Sambuelli, L., and Bava, S., 2012, Case study: A GPR survey on a morainic lake in northern Italy for bathymetry, water volume and sediment characterization: Journal of Applied Geophysics, v. 81, p. 48-56, doi:10.1016/j.jappgeo.2011.09.016.
Sambuelli, L., Calzoni, C., and Pesenti, M., 2009, Waterborne GPR survey for estimating bottom-sediment variability: A survey on the Po River, Turin, Italy: Geophysics, v. 74, no. 4, p. 1JA-Z73, doi:10.1190/1.3119262.
Sauck, W.A., and Seng, D.L., 1994, Near-shore sand thickness and stratigraphy mapping with
a submerged GPR antenna system; Southeast Lake Michigan (Abstract): Geological Society of America Abstracts with Programs, v. 26, no. 5, p. 59.
Van Dam, R.L., Hendrickx, J.M.H., Cassidy, N.J., North, R.E., Dogan, M., and Borchers, B., 2013, Effects of magnetite on high-frequency ground-penetrating radar: Geophysics, v. 78, no. 5, p. 1SO-Z134, doi:10.1190/geo2012-0266.1.